Body MRI sequences: A conceptual framework
Images
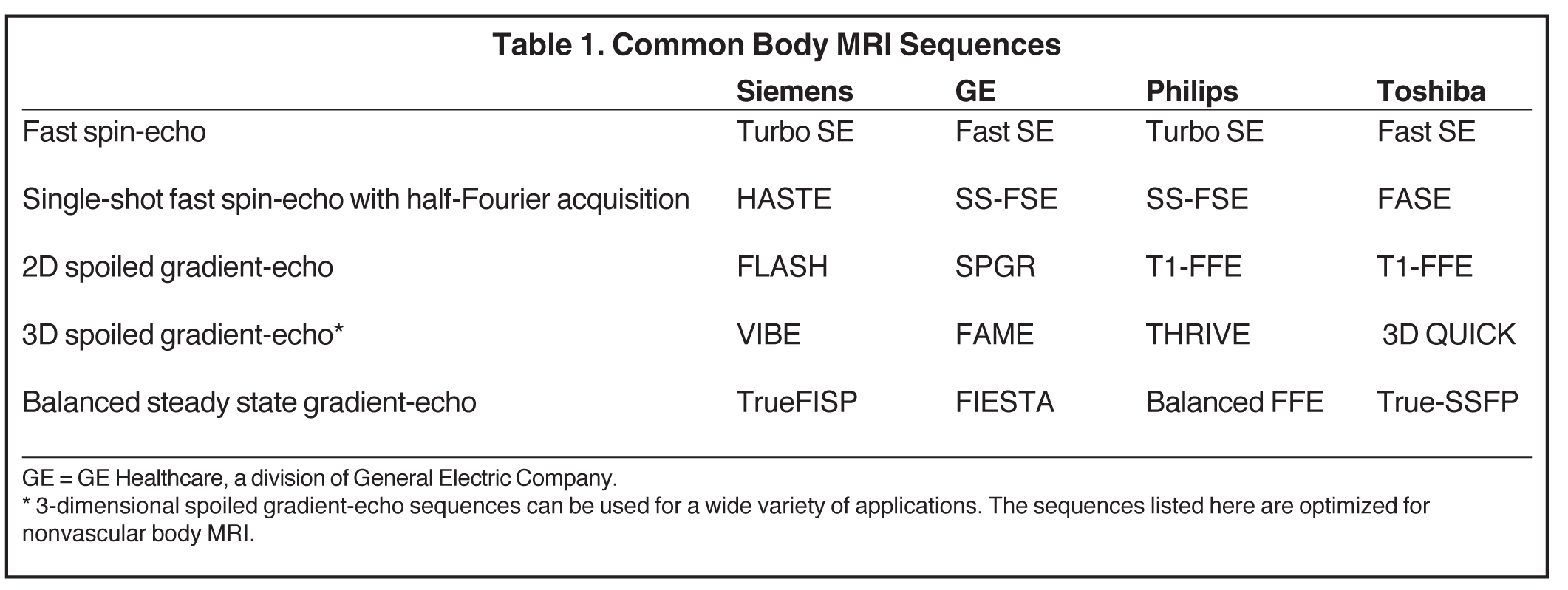
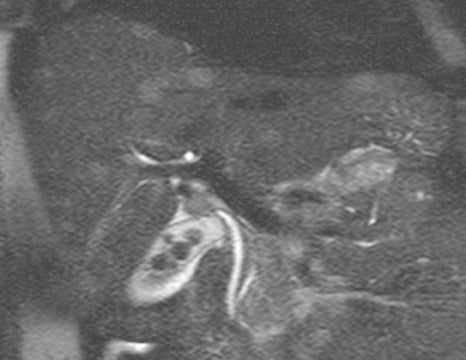
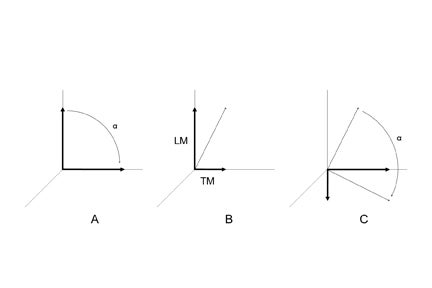
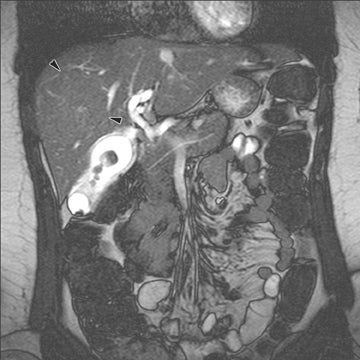
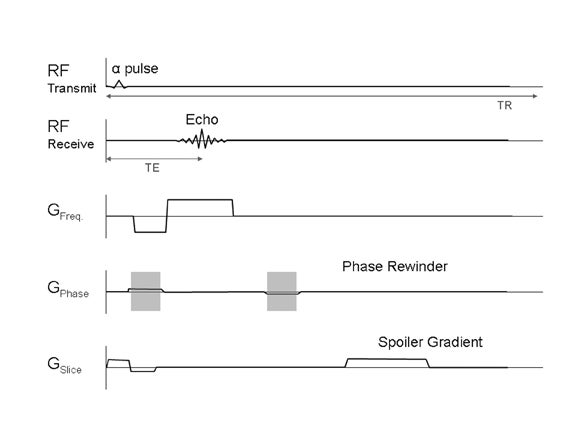
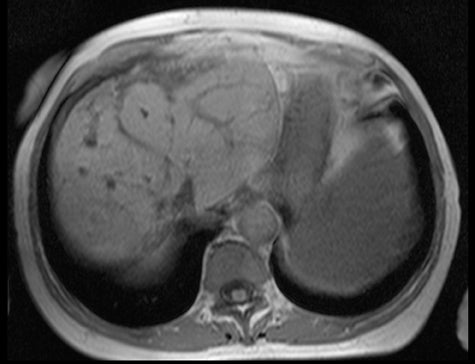
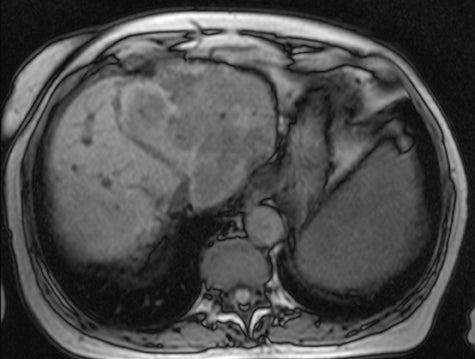
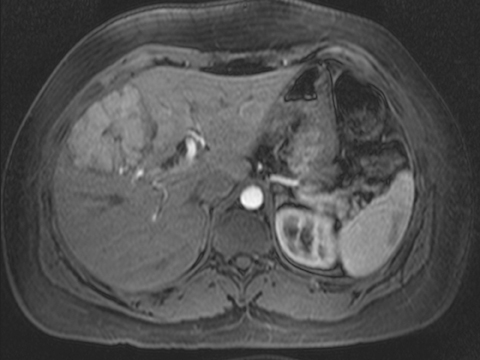
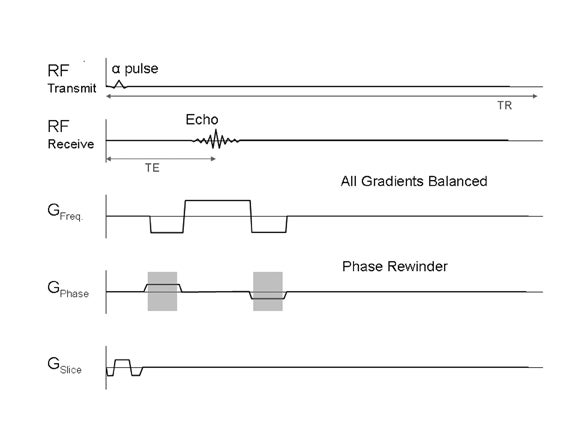
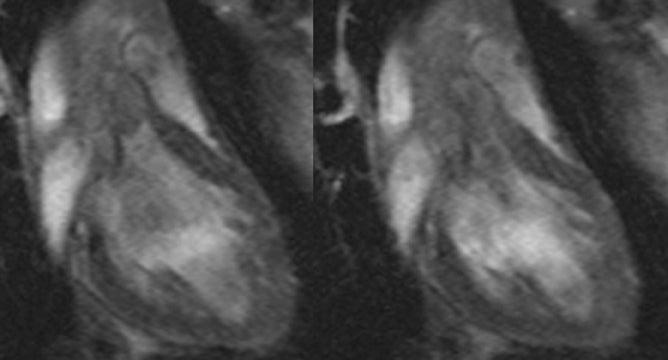
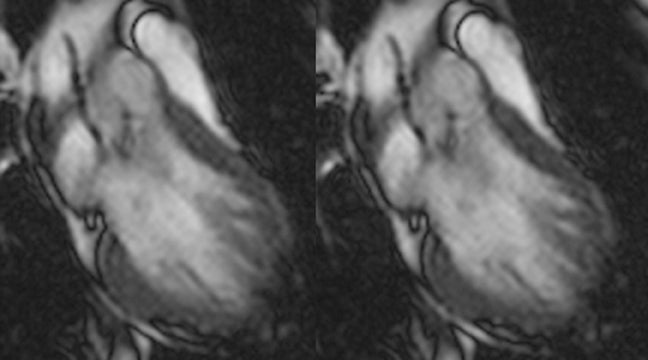
Dr. Lin is a Clinical Assistant Professor of Radiology, Department of Radiology at the University of Washington Medical Center and an Attending Physician at the Virginia Mason Medical Center, Seattle, WA.
Body magnetic resonance imaging (MRI) typically presents more challenges, from the perspective of imaging sequences, than MR imaging of the neurological and musculoskeletal systems. This is because to adequately image many of the organs, the patient must hold his breath, which limits the amount of time available for imaging, or the organ itself (the heart) is moving. Many of the sequences used in body MRI may be less familiar to radiologists than the sequences used in the neurological and musculoskeletal systems. The goal of this article is to present a broad conceptual and nontechnical framework that can serve as a basis for understanding these sequences. For the purposes of brevity, this article will assume a basic knowledge of the physics of and sequences employed in MRI.
In the author’s experience, a major barrier for radiologists learning body MRI sequences is the proliferation of trade names used by different manufacturers. The use of these trade names in the published literature may contribute to the confusion. However, the number of ways to generate an image in body MRI is actually limited, and the radiologist can avoid much of the potential confusion by thinking of the sequences generically.1 For example, the same 3-dimensional spoiled gradient-echo sequence will have numerous trade names attached to it by different manufacturers. However, referring to the sequence as a 3-dimensional spoiled gradient-echo sequence avoids potential confusion and is usually much more descriptive. That is, referring to a sequence generically tells us almost everything one needs to know about the sequence, while the trade names often give little information about the sequence other than a memorable catchphrase. Table 1 lists generic sequences and common trade names. The sequences we want to use should be fast, robust (meaning they generate diagnostic-quality images the majority of the time), and have the proper weighting.
Fast T2-weighted sequences
Let’s start with finding a T2-weighted sequence, which is fast, robust, and has the proper weighting. While there are many ways to increase the speed of a sequence once we have decided upon the sequence—eg, increasing bandwidth or decreasing repetition time (TR)—starting from the basic spin-echo sequence, there are 2 fundamental modifications that can be made. One of these is to eliminate the 180-degree refocusing pulse — that is, gradient-echo imaging. Without the refocusing pulse, the TR and echo time (TE) can be substantially decreased, which greatly decreases imaging time. However, for T2-weighted imaging, gradient-echo imaging presents several problems. Without the refocusing pulse, the images are now T2* rather than T2-weighted, and we no longer have the proper weighting. In addition, as relatively high TE values are needed for T2-weighted imaging, the T2* weighting will often result in substantial susceptibility artifacts and the sequence may no longer be robust. So it appears that in order to generate a robust, truly T2-weighted image, the 180-degree refocusing pulse should be retained.
The other basic modification to the spin-echo sequence that can be made is to acquire multiple lines of k-space during the TE interval. In a spin-echo sequence with a TE of milliseconds (msec), the time between 0 and 100 msec, when one line of k-space is filled, is “wasted” time in which nothing is being acquired. If 10 lines of k-space (an “echo train” of 10) are filled during this time (eg, at 10, 20, and up to 100 msec), the sequence can be completed 10 times as quickly. This is called fast spin-echo imaging. I will use this more common generic term, although it is also a trade name; a more generic but less familiar term for the same sequence is rapid relaxation with relaxation enhancement (RARE). How well does this work for T2-weighted imaging? Very well, as the retention of the 180-degree pulse gives us both T2-weighting and decreases susceptibility artifact, and the sequence can be very fast with a long-echo train. This technique can also be used for gradient-echo imaging. In a single shot, echoplanar gradient-echo sequence, all of the k-space is filled in one excitation. Echoplanar sequences tend not to be very robust.
Now it might be asked that if the length of the echo train determines the speed of the sequence, why not always use the longest echo train possible? One problem is that if we consider the previous example of a sequence with a TE of 100 msec and echo train of 10, each of the 10 lines of k-space filled actually has a different TE, ranging from 10 to 100 msec and thus a different signal intensity, and there is no actual TE for the sequence as a whole. In practice, the actual weighting of a fast-spin echo sequence is determined by the effective TE. As the center of k-space is important for signal-to-noise ratio and the periphery of k-space is important for image detail, the TE at which the center of k-space is obtained (the effective TE) will determine the weighting. However, there is still a problem with the 9 other lines of k-space, which contributes to image detail. As these are obtained at different TEs and will have different signal intensities, the image will be blurred. If the echo train is longer, the image blurring will increase as the number of different signal intensities increases.
Thus, increasing the echo train to decrease imaging time will typically increase image blurring. How important is this blurring? It depends on what is being imaged. Let’s consider fluid. Fluid has a long T2-relaxation time, so during a fast-spin echo sequence with a TE of 100 msec and an echo train of 10, there is actually little difference in the signal intensity of fluid between 10 and 100 msec, as there has not been much T2 relaxation in this time interval. Therefore, the effects of image blurring on fluid are minimal. This suggests that the use of very long echo trains to image fluid is optimal. On the other hand, solid tissue has a shorter T2-relaxation time, and the difference in signal intensity of solid tissue between 10 and 100 msec would be substantially greater than that of fluid. So image blurring in solid tissues might increase substantially at higher echo trains. During magnetic resonance cholangiopancreatography (MRCP) (Figure 1), a long echo train allows for very fast imaging of fluid, which is not substantially blurred. Solid tissue is substantially blurred, but this is not what is being primarily imaged. For other applications, shorter echo trains at the expense of imaging time may be desirable, if image detail of solid tissues is important.
A supplemental way of decreasing imaging time is the variety of techniques that rely on alternate ways of filling k-space.2 These are essentially shortcuts in k-space and decrease image time at the expense of signal-to-noise ratio (SNR). These techniques can be used to decrease imaging time in both fast spin-echo and gradient-echo imaging, and are best thought of in the framework of those sequences rather than in isolation. One class of techniques uses nontraditional trajectories for filling k-space, eg, spiral or radial. In another class of techniques, not all of the k-space lines are acquired and the remainder of k-space is filled with a partial Fourier method. Half-Fourier acquisition is a commonly used technique that relies on acquiring slightly more than half of the k-space lines and filling in the missing data using the conjugate symmetry of k-space. Fluid-imaging techniques, such as MRCP, typically will use this sequence: single-shot fast spin-echo with half-Fourier acquisition. If we learn the generic name of the sequence, it will tell us the essentials of the sequence. In a fast spin-echo technique, multiple lines of k-space are acquired in one excitation. A single-shot sequence is one in which all of the k-space is acquired in one excitation to maximize imaging speed and thus a large number of k-space lines must be filled. In order to accomplish this, a technique, half-Fourier acquisition, where only half of k-space is acquired is employed.
In summary, T2-weighted sequences in body MRI are typically performed with a fast spin-echo sequence. In these sequences, there is a trade-off between imaging time and image blurring, which is determined by the echo train. Very fast T2-weighted images can be obtained using a single-shot technique. Additional k-space shortcuts can be used with fast spin-echo sequences, which further decrease imaging time at the expense of SNR.
Fast T1-weighted sequences
If we want to acquire a fast, robust study with T1-weighted image contrast, one option is a fast spin-echo sequence, as used for T2-weighted imaging. However, this does not work nearly as well as with T2-weighted imaging. The substantially shorter TEs needed for T1-weighted image contrast limit the increase in acquisition time possible. For example, with a T1-weighted sequence with a TE of 20 milliseconds, only 2 lines of k-space might be filled during 20 milliseconds. This would halve the imaging time, but is still not nearly enough for breath-hold imaging.
Therefore, gradient-echo imaging must be used to acquire fast, robust, T1-weighted images. However, the requirement for speed now presents a problem. Fast gradient-echo sequences need to use a short TR. If the TR is shorter than the T2-relaxation times of the imaged tissues, which will typically be case for breathhold imaging, the transverse magnetization will not fully decay before the next radiofrequency (RF) pulse. After multiple RF pulses, there will be a “steady-state” residual-transverse magnetization,3 which is constant (Figure 2). This residual-transverse magnetization contributes T2-weighting to the image. The sequence is now fast and robust, but it no longer has strict T1-weighting.
More specifically, we have a steady-state, or coherent, sequence in which the image contrast depends upon the T2- to T1-ratio. What does this actually look like, and is this a positive or negative factor? Fat and fluid will have high signal, as fat and fluid have comparable T1- and T2-relaxation times. Other tissues will have substantially lower signals, as T2-relaxation times are much shorter than T1-relaxation times. So we will obtain images where fat and fluid is bright, and everything else is isointense (Figure 3). This isn’t the type of image contrast needed for most body MRI applications.
Steady-state contrast is thus the default condition for fast gradient-echo imaging. To remove this contrast, the TR could be increased so the transverse magnetization will relax fully after each RF pulse. However, the sequence would no longer be fast enough for breathhold imaging. Instead, the residual-transverse magnetization is removed through “spoiling,”4 which can be done with a gradient or RF pulse (Figure 4). Removal of the residual transverse magnetization does result in a fast, robust sequence, which is T1-weighted.
Thus the majority of fast T1-weighted sequences in body MRI applications are spoiled gradient-echo images. These can be acquired 2-dimensionally or 3-dimensionally (in MRI, 3-dimensional means that there is phase-encoding in 2 axes). Often chemical shift (in- and out-of-phase) imaging is used in conjunction (Figure 5). For applications in which a very short acquisition time is needed (eg, MR angiography) these techniques are usually combined with a k-space shortcut.
The 3-dimensional spoiled gradient-echo sequence is a versatile sequence that can be used both for MR angiography and imaging of the parenchymal organs (Figure 6), simply by changing the flip angle. The Ernest angle5 is the flip angle in spoiled gradient-echo imaging where signal intensity is maximized:
cos α = exp (-TR/T1).
As the T1-relaxation time of intravascular contrast is shorter than that of a parenchymal organ, the 3-dimensional spoiled gradient-echo sequence would use a larger flip angle for MR angiography and a smaller flip angle for parenchymal organ imaging. The flip angle must also be adjusted for the TR (the higher the TR, the greater the flip angle).
Magnetization-prepared gradient-echo sequences are another way to obtain fast T1-weighted sequences that will not be addressed here as these sequences are not commonly used for body MRI. In these sequences, the T1 weighting is provided by a preparation pulse. Typically these sequences are used to obtain multiple rapid T1-weighted images at the same level; eg, for myocardial perfusion.
In summary, the spoiled gradient-echo sequence is the workhorse sequence for fast T1-weighted imaging. Fast gradient-echo sequences, without additional modification, are in the steady-state and have T2/T1 weighting; they must be spoiled to obtain T1-weighting. Spoiled gradient-echo sequences can be modified for a variety of applications by adjusting the flip angle.
Steady-state sequences
Let’s return to the steady-state gradient-echo sequence. This sequence is both fast and robust. It has higher SNR than a spoiled gradient-echo sequence, as the unspoiled residual-transverse magnetization contributes to the signal. The intrinsically high SNR can be parlayed into a faster imaging time by increasing the bandwidth and decreasing the TR. So what’s not to like about a fast sequence with high SNR? We also need the correct image contrast, and T2/T1-image contrast is not the contrast needed for most body imaging applications. In the past, sequences were always designed to avoid T2/T1 contrast. However, with increased gradient strengths and shorter imaging times, there were applications in which strict T1 or T2 weighting was not needed, as long as imaging could be performed quickly. A good example is cine cardiac imaging, in which what is required for image contrast is not strict T1 or T2 weighting but simply that the blood appears much brighter than the myocardium. So once MRI scanners were fast enough, steady-state sequences, which were previously explicitly avoided, turned out to be very useful.
There are a few different steady-state sequences.1 These are designed to maintain the steady state rather than spoil it. Some of these sequences have minimal clinical use, others have specialized applications (eg, DESS or CISS). The relevant steady-state sequence for body MRI applications is a fully refocused or balanced sequence, in which the steady state is maintained with balanced gradients that minimize dephasing (Figure 7).
Steady-state sequences have essentially replaced spoiled gradient-echo sequences for cine cardiac imaging in recent years. Steady-state sequences are faster, and have higher SNRs and contrast-to-noise ratios. With a spoiled sequence, the “bright blood” comes from through plane-flow enhancement (similar to time-of-flight angiography). Steady-state gradient-echo sequences are not flow-sensitive, and the “bright blood” comes from intrinsic contrast. There are substantial effects of this change in sequences. For example, with steady-state gradient-echo sequences, the myocardium can be better delineated from the cardiac chamber (Figure 8). This affects determination of ventricular volumes6 and thus ejection fraction (ventricular volumes determined by steady-state sequences are larger than those determined by spoiled sequences). However, not everything is advantageous. As steady-state sequences are not flow-sensitive, it is harder to visualize turbulent flow (eg from regurgitation) (Figure 9).
Steady-state sequences can also be used to visualize blood outside of the heart; that is, for nonenhanced-MR angiography. This may be more relevant with the recently reported relationship between gadolinium and nephrogenic systemic fibrosis. Steady-state sequences are the most common sequences used to obtain “scout” images in body MR imaging. As very fast sequences with high SNR, they are optimally suited for this purpose. As steady-state sequences are very fast sequences where fluid is bright, they can be viewed as a complement or alternative to single-shot fast spin-echo sequences for body MRI applications (eg, for MRCP or fetal imaging). Single-shot fast spin-echo sequences are typically preferred for most of these applications, but one advantage of steady-state sequences is substantially lower radiofrequency deposition.
In summary, steady-state gradient-echo sequences have become the standard for cine cardiac imaging, and have other applications as well. These sequences are very fast and robust, but they are limited to applications where T2/T1-image contrast is acceptable.
REFERENCES
- Boyle GE, Ahern M, Cooke J, et al. An interactive taxonomy of MRI sequences. Radiographics. 2006;26:e24.
- Lee VS. Fast scanning and k-space shortcuts. In: Lee VS. Cardiovascular MRI: Physical principles to practical protocols. Philadelphia, PA: Lippincott Williams and Wilkins. 2006:138-158.
- Chavan GB, Babyn PS, Jankharia BG, et al. Steady-state MR imaging sequences: Physics, classification, and clinical applications. Radiographics. 2008;28:1147-1160.
- Mitchell DG, Cohen MS. Pulse sequences: Gradient echo and spin echo. In: MRI Principles, 2nd Edition. Philadelphia, PA: Elsevier Science; 2003:400.
- Mitchell DG, Cohen MS. MRI principles. Philadelphia, PA: Saunders; 2004:163-176.
- Mitchell DG, Cohen MS. Proton environments and T1 relaxation. Philadelphia, PA: Saunders; 2004:177-185.
- Mitchell DG, Cohen MS. MRI principles. Philadelphia, PA: Saunders; 2004:21-34.
- Hudsmith LE, Petersen SE, Tyler DJ, et al. Determination of cardiac volumes and mass with FLASH and SSFP cine sequences at 1.5 vs 3 tesla: A validation study. J Magn Reson Imaging. 2006;24:312-318.